Author: Afreen Hossain
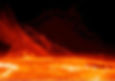
Abstract
The study "Understanding And Predicting Solar Flares: The Sun's Explosive Events" concludes with a thorough analysis of solar flares, their cause, consequences, and importance. It emphasizes on the importance of researching these occurrences in order to improve forecasting skills, protect technology and infrastructure, and deepen our understanding of the Sun and the cosmos in general. The study of solar flares is depicted as crucial for understanding both stellar physics and astrophysics. Solar flares offer insights into complex plasma processes and magnetic fields in astrophysical environments, aiding our comprehension of how stars function.
Introduction
Solar flares are fascinating and powerful events that occur on the Sun, our nearest star. The Sun is a massive ball of hot gas primarily composed of hydrogen and helium, and it generates energy through nuclear fusion in its core. This energy is constantly being released in the form of light and heat, which are essential for supporting life on Earth. But occasionally the Sun experiences sudden releases of energy in the form of solar flares.
To understand solar flares better, let's first explore the Sun's structure and its magnetic field. The Sun's structure can be divided into several layers, each with distinct characteristics and behaviors, starting from the core, which experiences immense pressure. The energy generated by nuclear fusion is carried outward through the radiative zone. In the radiative zone, the energy produced in the core slowly diffuses through a sea of ionized atoms, mainly hydrogen and helium, in the form of electromagnetic radiation. This process can take thousands to millions of years as the photons interact with and scatter off particles along their journey to the Sun's surface. Imagine this process as a slow and steady wave propagating through calm waters. The photons are like little ripples carrying energy from the depths of the Sun towards its outer layers.
The Sun consists of several layers: the core radiative zone, convective zone, photosphere, chromosphere, and corona. The core is the central region where nuclear fusion reactions occur, converting hydrogen into helium and releasing a tremendous amount of energy.
The Sun's magnetic field, which plays a crucial role in its activity, is generated by the motion of charged particles within the Sun, particularly through a process called the solar dynamo. The Sun's interior undergoes differential rotation, causing the magnetic field lines to twist and tangle over time. This process leads to the formation of Sunspots and active regions on the Sun's surface. Sunspots are dark, cooler areas on the Sun's visible surface, and they are closely associated with the Sun's magnetic activity. The intense magnetic fields in Sunspots can become unstable, leading to solar flares. When a solar flare occurs, there is a sudden release of magnetic energy that has been stored in the Sun's atmosphere. The energy is released in the form of electromagnetic radiation, including x-rays and extreme ultraviolet light. These emissions can be up to a million times more energetic than the light emitted by the Sun during its normal activity. The process leading to a solar flare starts when the Sun's magnetic field lines become twisted and stressed. This can happen when magnetic fields of opposite polarity come close together causing a magnetic reconnection event. During this reconnection, the magnetic energy is converted into kinetic and thermal energy accelerating charged particles and heating the surrounding plasma as the magnetic energy is released. It propels energetic particles away from the flare site. Some of these particles can reach the Earth producing beautiful auroras in the polar regions. These auroras are caused when charged particles interact with the Earth’s magnetic field and atmosphere creating a glowing display of colors in the night sky.
Solar flares are classified based on their X-ray intensity into categories such as B, C, M, and X, with X-class flares being the most powerful. They can last from a few minutes to several hours depending on their intensity and complexity, despite their distance from Earth. Solar flares can have significant impacts on our technology and communication systems.
Significance of solar flares
Solar flares are of great importance due to their significant impact on Earth's atmosphere and technology. The most common effects of solar flares include radio blackouts. The intense radiation from solar flares can disrupt radio communications, especially at high frequencies, making it difficult to communicate with aircrafts, ships, and other ground stations. Geomagnetic storms can also occur as solar winds interact with the Earth's magnetic field, causing disruptions in power grids, satellite damages, and GPS signal interference.
Additionally, the radiation from solar flares can increase the risk of cancer and other health problems for astronauts and people in high-altitude aircrafts. Indirectly, solar flares can also cause changes in Earth's climate, such as the aurora borealis appearing further south than usual and affecting weather patterns. As our society becomes increasingly reliant on technology, understanding the risks posed by solar flares and taking steps to mitigate them is crucial. Developing better forecasting tools and hardening our infrastructure against the effects of solar storms are necessary measures.
Importance of studying solar flares
The study of solar flares is crucial due to their significant impact on our planet and space environment. Solar flares are crucial to our understanding of stellar physics and astrophysics. These eruptions result from complex magnetic interactions within the Sun's outer layer. By studying solar flares, researchers can gain deeper insights into fundamental plasma processes and magnetic fields in astrophysical environments. This knowledge would provide valuable insights into how stars like the Sun function and how magnetic energy is converted into kinetic energy and radiation.
Solar flares have a profound impact on space weather, which directly affects Earth and its surrounding space environment. During a solar flare, enormous amounts of high-energy particles and radiation are released. These particles can create disturbances in the ionosphere, which can interfere with radio communications and GPS signals. Understanding the processes behind solar flares is crucial for accurately predicting and mitigating these potential disruptions. Furthermore, solar flares can damage the electronics of satellites, leading to malfunctions or complete failures. By studying solar flares, scientists can develop better strategies to protect our technology and astronauts from the harmful effects of these eruptions. Additionally, solar flares are linked to coronal mass ejections (CMEs) - massive clouds of solar material and magnetic fields ejected into space. When these CMEs interact with Earth's magnetosphere, they can trigger geomagnetic storms. These storms can induce electric currents in power lines, pipelines, and other long conductive structures, potentially causing widespread blackouts and damage to electrical grids. By studying solar flares and their associated CMEs, scientists can improve space weather forecasting models and provide early warnings to help governments and industries prepare and protect critical infrastructure. Moreover, solar flares have implications beyond our planet. They can be observed in other stars, providing valuable data for understanding stellar activity and the potential habitability of exoplanets. Studying solar flares in distant star systems helps us assess the conditions on exoplanets and the likelihood of supporting life.
As we continue to explore and utilize space for scientific and commercial purposes, understanding solar flares remains a vital area of research with far-reaching implications for our society and the future of space exploration.
What are CMEs?
Coronal mass ejections, also known as CMEs, are ejections of plasma and magnetic field that occur in the Sun's corona. They are the most energetic and largest events that happen on the Sun, and they can significantly affect the technology and atmosphere here on Earth. CMEs result in a sudden release of energy in the Sun's atmosphere, caused by the snapping back of magnetic field lines together. This snapping back causes huge amounts of plasma and magnetic fields to be ejected into space. CMEs can travel at speeds of up to one million miles per hour, and when they reach Earth, they can interact with the planet's magnetic field, causing geomagnetic storms. CMEs occur most frequently during the Sun's solar cycle, which lasts around 11 years. The Sun's activity peaks every 11 years, and during this time, CMEs are more common and more powerful.
What is Earth's magnetosphere?
The Earth's magnetosphere is a space area around our planet that is controlled by its magnetic field. It serves as a protective shield against harmful solar radiation and particles, and it also helps regulate the atmosphere. The magnetosphere's shape is influenced by the solar wind, which is a stream of charged particles emanating from the Sun. The solar wind flows past the Earth at speeds of up to one million miles per hour. When the solar wind encounters Earth's magnetic field, it is deflected, creating a bubble-like region of space that is protected from the solar wind.
How does studying solar flares in distant star systems help us assess the conditions on exoplanets and the likelihood of supporting life?
Solar flares are powerful bursts of energy that can release large amounts of radiation. If an exoplanet is located within the habitable zone of its star, it is likely to be exposed to solar flares. The amount of radiation that an exoplanet receives from solar flares can affect its atmosphere and surface. If the radiation is too high, it can damage the atmosphere and make it difficult for life to exist. By studying solar flares in distant star systems, we can learn more about the potential hazards that exoplanets face. This information can help us to identify exoplanets that are more likely to be habitable. For example, if we know that a star is producing a lot of solar flares, we can estimate how much radiation an exoplanet orbiting that star would be exposed to. If the radiation level is too high, we can then rule out that exoplanet as a potential home for life. We can also study the composition of exoplanet atmospheres to learn more about how they interact with solar flares. Some molecules in the atmosphere, such as ozone, can absorb harmful radiation from solar flares. If an exoplanet has a thick ozone layer, it is more likely to be able to protect its surface from harmful radiation.
We can also study the magnetic fields of exoplanets to learn more about how they protect their atmospheres from solar flares. The magnetic field of a planet can deflect solar wind and solar flares just like the Earth, helping to protect the atmosphere from harmful radiation. If an exoplanet has a strong magnetic field, it is more likely to be able to protect its atmosphere from solar flares. By combining all of this information, we can get a better understanding of the potential habitability of exoplanets. This information can help us to identify exoplanets that are more likely to be home to life.
Structure of the Sun
The core of the Sun is the central and most crucial region where the incredible energy that powers our star is generated. It is an extraordinarily hot and dense region, with temperatures reaching about 15 million degrees Celsius (27 million degrees Fahrenheit) and pressures millions of times greater than that of the Earth's surface at these extreme conditions. Nuclear fusion reactions such as the proton-proton chain reaction take place here. In this process, hydrogen nuclei combine to form helium nuclei, releasing an enormous amount of energy in the form of gamma rays. The core acts as a natural nuclear reactor, converting mass into energy according to Einstein's famous equation (Energy)=(mass)X(the square of the speed of light in vacuum). These high-energy gamma rays are continuously produced in the core, but they cannot travel freely through the dense plasma; instead, they undergo multiple scattering interactions with the charged particles in the core. This process is known as radiative diffusion, and it significantly slows down the movement of photons through the core. It can take thousands to millions of years for a single photon to make its way from the core to the surface of the Sun due to the vast number of scattering events. The intense pressure generated by the high temperature in the core counteracts the gravitational force, maintaining the Sun's size and shape. The energy produced in the core is the primary source of Sunlight and heat that sustain life on Earth. It is also the driving force behind the Sun's magnetic activity and solar phenomena such as Sunspots, solar flares, and coronal mass ejections. Understanding the core is essential for predicting and understanding solar behavior, space weather, and the Sun's overall influence on our planet and the space environment. Studying the core of the Sun provides scientists with valuable insights into fundamental nuclear physics, astrophysics, and the processes that govern the life cycle of stars. However, due to its incredibly high temperature and density, direct observations of the core are not possible. Instead, researchers use sophisticated models and simulations to gain a deeper understanding of this enigmatic and essential region at the heart of our solar system.
The radiative zone is a crucial region within the Sun that plays a fundamental role in transporting energy from its central core to the outer layers, eventually reaching the photosphere (the layer that emits the light which we see as Sunlight is called the photosphere - it is the visible surface of the Sun) and being radiated into space as Sunlight. This zone lies between the core and the convective zone, making up a significant portion of the Sun's interior. The radiative zone is a region of extremely high temperature and pressure where energy is carried primarily by photons through a process known as radiative diffusion. The journey of energy through the Sun starts at the core, where nuclear fusion reactions release an enormous amount of energy in the form of high-energy gamma rays. These gamma rays are absorbed and re-emitted by the charged particles (protons and electrons) present in the core's dense plasma. Due to the density and opacity of the material in the core, the gamma rays experience multiple scattering interactions before they can make their way out. As the gamma rays move outward through the core, they lose energy with each scattering event. This loss of energy causes their wavelength to increase, transforming them into lower-energy photons, mainly in the form of X-rays and ultraviolet radiation. This process continues until the photons energy reaches a level where they can escape the core without being reabsorbed. Once the photons reach the boundary of the core and the radiative zone, they encounter a less dense region. Here, the plasma still absorbs and emits photons, but the mean free path, the average distance a photon travels before being scattered is much larger compared to the core; consequently, photons can travel longer distances between scattering events. Radiative diffusion is the primary mechanism by which energy is transported through the radiative zone. This process involves the continuous absorption and re-emission of photons by the charged particles in the plasma. When a photon is absorbed by an atom or ion, it excites an electron to a higher energy level. The excited electron rapidly returns to its original state due to instability, releasing the absorbed energy as a new photon with the same energy but possibly a different direction and phase. The photons in the radiative zone perform a random walk pattern, moving in a zigzag fashion as they undergo countless scattering interactions. This random motion results in a net movement of energy outward from the core to the Sun's surface; however, it is important to note that this energy transport process is relatively slow compared to other layers of the Sun as the mean free path of photons in the radiative zone is still quite short. The density of the plasma decreases with increasing distance from the core. This means that the average distance between particles increases, resulting in fewer scattering interactions for the photons. Consequently, the photon's mean free path becomes longer as they travel further into the radiative zone. The energy transport time through the radiative zone is quite lengthy; it can take thousands to millions of years for a photon to traverse this region before reaching the convective zone. The slow pace of energy transport is a consequence of the high density and opacity of the core and radiative zone materials. In contrast, the convective zone, which lies above the radiative zone, allows for more rapid energy transport due to its convective currents. The transition between the radiative zone and the convective zone is known as the tachocline. It is a region of significant interest for scientists as it may hold clues to understanding the Sun's magnetic field generation and its dynamo processes. Observations of the radiative zone are challenging due to its inaccessible location deep within the Sun's interior. As a result, scientists rely on sophisticated solar models and simulations based on our understanding of nuclear physics, plasma physics, and thermodynamics to study the behaviour of this critical region.
Basically, in the radiative zone of the Sun the energy produced in the core tries to make its way out towards the surface but it's not an easy journey because the core is dense and hot. The energy in the form of gamma rays gets absorbed and scattered many times by the charged particles. As the energy moves outward from the core, it enters the radiative zone where the material is still dense but less so than the core. Here the energy takes a longer, more indirect path. The energy is in the form of photons (tiny packets of light), and they bounce around like a pinball getting absorbed and re-emitted by the particles in the plasma. The photons continue this random bouncing until they finally reach the surface layer of the Sun called the photosphere. At the photosphere the material becomes less dense, and the photons can travel more easily. They escape the Sun's surface and travel through space as Sunlight. This process of energy transport through the radiative zone is slow and can take a long time for the energy to reach the surface, but this is how the Sun shines and provides light and warmth to us here on Earth.
Why is the core layer's density greater than the radiative layer?
The core is incredibly hot and dense, with temperatures reaching around 15 million degrees Celsius (27 million degrees Fahrenheit) and pressures millions of times greater than that of the Earth's surface. The core is the primary source of the Sun's energy; in contrast, the radiative zone lies above the core but is still very hot. The radiative zone is less dense compared to the core because as the energy, in the form of photons, moves outward from the core, it encounters a region where the plasma is not as tightly packed. This decrease in density occurs because the high temperature in the core drives the gas to expand. The plasma in the core of the Sun is incredibly hot and dense. The temperature is so high that the hydrogen atoms are stripped off their electrons and become free-floating protons and electrons. This plasma is under enormous pressure, and the high temperature and pressure cause the plasma to expand, and as a result, the material becomes less dense as you move away from the core. The lower density in the radiative zone allows the photons to travel longer distances between interactions with charged particles. The average distance a photon travels before scattering, increases in the radiative zone compared to the core. This means that the photons experience fewer scattering events per unit distance in the radiative zone, making it easier for them to move through this region.
The convective zone is a vital layer in the interior of the Sun, situated between the radiative zone and the photosphere. It plays a crucial role in transporting energy from deeper layers to the Sun's visible surface (photosphere). Instead of relying on radiative diffusion like the radiative zone, the convective zone uses convection to operate. The convective zone is characterized by its convective currents, which are large-scale movements of plasma caused by high temperatures at the base of the zone. The plasma becomes less dense and buoyant, rising towards the Sun's surface and carrying energy with it. As it cools and becomes denser, it sinks back down to the deeper layers in a cycle similar to boiling water. These convective currents create cellular patterns on the Sun's surface known as granules, which appear as bright hot regions at their centers and darker cooler regions at their edges. Convection is a more efficient mode of energy transport than radiative diffusion, allowing the energy to move more rapidly from the core towards the surface. As seen before, the transition layer between the radiative zone and the convective zone is called the tachocline, where energy transport changes from radiative diffusion to convection. This region is important in generating the Sun's magnetic field and the solar dynamo process. The photosphere is the visible surface of the Sun and the layer where Sunlight is emitted into space. It is the innermost layer of the Sun that we can observe directly. The temperature of the photosphere is around 5500 degrees Celsius, making it the coolest layer of the Sun. The density is much higher in this layer, allowing light to be absorbed and re-emitted multiple times before escaping into space. (The charged particles in the plasma in the photosphere can absorb photons of light emitted from the lower layers of the Sun, such as the core and the radiative zone. An opaque material does not allow light to pass through it easily; instead, it absorbs and scatters light. This causes the photosphere to appear opaque and gives the Sun its familiar yellowish-white appearance. The photosphere is marked by various solar features, including Sunspots, which are regions with strong magnetic fields that inhibit convection and cause the surface temperature to drop. The photosphere's convective motion creates granules and super granules, which are small cellular-like structures visible on the Sun's surface. The photosphere is typically not visible to the naked eye due to the extreme brightness of the Sun’s outer layers. However, during a total solar eclipse, what is visible is the corona.
The corona is a layer of the Sun's atmosphere that extends far into space. It is made up of extremely hot and tenuous plasma with temperatures reaching several million degrees Celsius. Despite being farther away from the Sun's core, the temperature of the corona is much higher than the photosphere. This temperature inversion is known as the coronal heating problem and the exact mechanism responsible for heating the corona to such high temperatures is not yet fully understood. It is likely related to the Sun's magnetic fields and wave interactions. The corona is the source of the solar wind, which is a continuous flow of charged particles that mostly consists of electrons and protons. These particles escape the Sun's gravitational pull and stream out into space. The solar wind affects the space environment around our solar system, interacting with Earth's magnetic field and creating space weather phenomena. Typically, the corona is not visible due to the extreme brightness of the photosphere. However, during a total solar eclipse, when the moon blocks out the photosphere, the corona becomes visible as a faint pearly-white halo around the Sun. Total solar eclipses provide an opportunity for scientists to study the corona and learn more about its structure and behavior. The corona is also associated with coronal mass ejections (CMEs).
On predicting solar flares
Magnetohydrodynamics (MHD): Imagine you have a pot of boiling water on a stove. The bubbles rising in the water are like the plasma, and the steam above the water is like the magnetic field. In this analogy, MHD equations help us understand how the bubbles move, how they affect the steam, and how the steam, in turn, influences the bubbles. The MHD equations are basically a set of rules that describe how the plasma and magnetic fields change. They include equations for things like:
Conservation of Mass: This is like saying that the total amount of plasma in a specific region doesn't change; it just moves around.
In a solar flare, the Sun becomes very active, like a big bubbling pot of hot soup. This soup is made of tiny, charged particles - plasma.
The conservation of mass equation is like a rule that says, "The total amount of soup (plasma) in the pot stays the same. It doesn't magically appear or disappear."
The conservation of mass equation, also known as the continuity equation, is:
∂ρ/∂t + ∇·(ρV) = 0
- ∂ρ/∂t: This part means, "How much amount of soup (plasma) changes over time." If it is positive, it means more particles in soup are added, and if it's negative, it means some soup is removed.
- ∇·(ρV): This part looks at how the soup moves around inside the pot. It's like checking how much soup flows in and out of a specific area.
The equation simply tells us that the rate at which the amount of the soup (plasma) changes over time and the flow of soup in and out of a particular area must balance out. If we add some soup to one part of the pot, some other soup must move away to keep the total amount of soup the same. Therefore, the total should remain unchanged, and hence, the change is equated to zero.
For solar flares, this equation helps scientists understand how the amount of plasma changes during the flare. It helps them study how the hot plasma moves and behaves during the exciting explosion on the Sun.
The symbols in the equations are pronounced as follows:
- ∂ρ/∂t is the partial derivative of 'rho'(ρ) (which is the density of the plasma) with respect to time.
- ∇·(ρV): This is pronounced as "del dot rho times V." The symbol ∇ (pronounced "del") is called the nabla operator, and it represents a special tool used in mathematics to measure changes and movements in a space. The dot (·) means we are taking the dot product of ρ and V. "Rho" (ρ) again represents the density of the plasma (amount of soup), and "V" represents the velocity (how fast the soup moves). So, "∇·(ρV)" means "looking at how the soup moves around inside the pot."
Conservation of Momentum: This equation deals with how the plasma gets pushed or pulled due to forces, such as the pressure inside the plasma, the gravity, and the magnetic forces. This equation represents the conservation of momentum in the plasma:
ρ(∂V/∂t + V·∇V) = -∇P + (1/μ₀)(B·∇)B + ρg
Imagine you have a balloon filled with air, and you release it in an open field. As the balloon flies around, its motion is affected by various forces, such as wind, gravity, and internal pressure. The conservation of momentum equation helps us understand how these forces influence the balloon's movement.
- ρ: In the context of solar flares, ρ represents the density of the plasma in the Sun's atmosphere. It tells us how much mass is present per unit volume in the region where the flare occurs.
- V: V represents the velocity of the plasma. During a solar flare, charged particles (plasma) are accelerated and ejected from the Sun's surface at high speeds.
- ∂V/∂t: This term represents how the velocity of the plasma changes over time during the flare. Solar flares involve rapid changes in plasma velocities due to the release of magnetic energy.
- V·∇V: This term describes how the plasma accelerates or decelerates in different directions. It helps us understand how the magnetic forces influence the movement of plasma during a solar flare.
- -∇P: The negative gradient of the plasma pressure (P) represents the force due to pressure differences within the solar atmosphere. During a solar flare, the release of magnetic energy causes sudden changes in pressure, contributing to the dynamics of the flare.
- (1/μ₀)(B·∇)B: This term involves the magnetic field vector (B) and its gradient (∇). Solar flares are driven by magnetic reconnection, where magnetic field lines rearrange and release energy. This term describes how magnetic forces influence the plasma's behaviour during this process.
- ρg: In the context of solar flares, ρg represents the force due to gravity acting on the plasma in the Sun's atmosphere. While gravity is generally weaker than other forces during solar flares, it may still have some effect on the plasma dynamics.
Applying the conservation of momentum equation to our balloon example:
ρ(∂V/∂t + V·∇V) = -∇P + ρg
This equation tells us that the change in momentum of the air inside the balloon is equal to the sum of forces acting on it:
- The left side (ρ(∂V/∂t+V·∇V)) describes how the momentum of the air changes due to its acceleration and the effect of the wind on the balloon's motion.
- The right side (-∇P+ρg) describes the forces acting on the air inside the balloon. The pressure difference inside the balloon (the -∇P term) and the force of gravity (the ρg term) are the forces influencing the balloon's movement.
So, in this example, the conservation of momentum equation helps us understand how the velocity and direction of the balloon change over time due to the combined effects of wind, pressure, and gravity.
Conservation of Energy: This tells us that the energy in the plasma changes over time, considering things like heating and cooling effects.
Magnetic Induction Equation: This explains how the magnetic field changes as the plasma moves and behaves. It helps us understand how the magnetic field lines move and evolve.
The MHD equations help scientists predict and understand solar flares. By studying these equations and using computer simulations, scientists can learn more about the Sun's behavior and its influence on space weather, which affects things like satellites, GPS systems, and even power grids on Earth. Understanding solar flares is essential for our technological society to be prepared and protected from their effects.
Collecting Solar Flares:
One theoretical concept involves the idea of capturing and studying particles from the solar flares that erupt from the Sun. The notion revolves around experimentation with the characteristics and potential harmfulness of these solar emissions once they interact with Earth's environment. However, it is vital to acknowledge that solar flares discharge an immense amount of energy and particles into space, equivalent to the energy of billions of atomic bombs. Successfully capturing and safely controlling such high-energy particles would necessitate technologies that are well beyond our current capabilities.
Satellite-Based Monitoring Theory:
In a proposed satellite-based monitoring approach, satellites are poised to become pivotal tools for vigilantly observing the Sun's activities and their influence on Earth's space environment, often referred to as space weather.
The satellite-based monitoring strategy allows for the detection of anomalies in the Sun's behavior, such as sudden spikes in X-ray or ultraviolet radiation. These anomalies can serve as early warning indicators of impending solar flares. Consequently, this approach would empower us to implement measures to safeguard critical technology and infrastructure from potential repercussions linked to space weather events.
References
Asplund, M., Grevesse, N., Sauval, A.J. and Scott, P., 2009. The chemical composition of the Sun. Annual review of astronomy and astrophysics, 47, pp.481-522.
Stix, Michael. The Sun: an introduction. Springer Science & Business Media, 2004.
Svestka, Zdenek. Solar flares. Vol. 8. Springer Science & Business Media, 2012.
Fletcher, L., Dennis, B.R., Hudson, H.S., Krucker, S., Phillips, K., Veronig, A., Battaglia, M., Bone, L., Caspi, A., Chen, Q. and Gallagher, P., 2011. An observational overview of solar flares. Space science reviews, 159, pp.19-106.